How the Solar System led planet-hunters astray
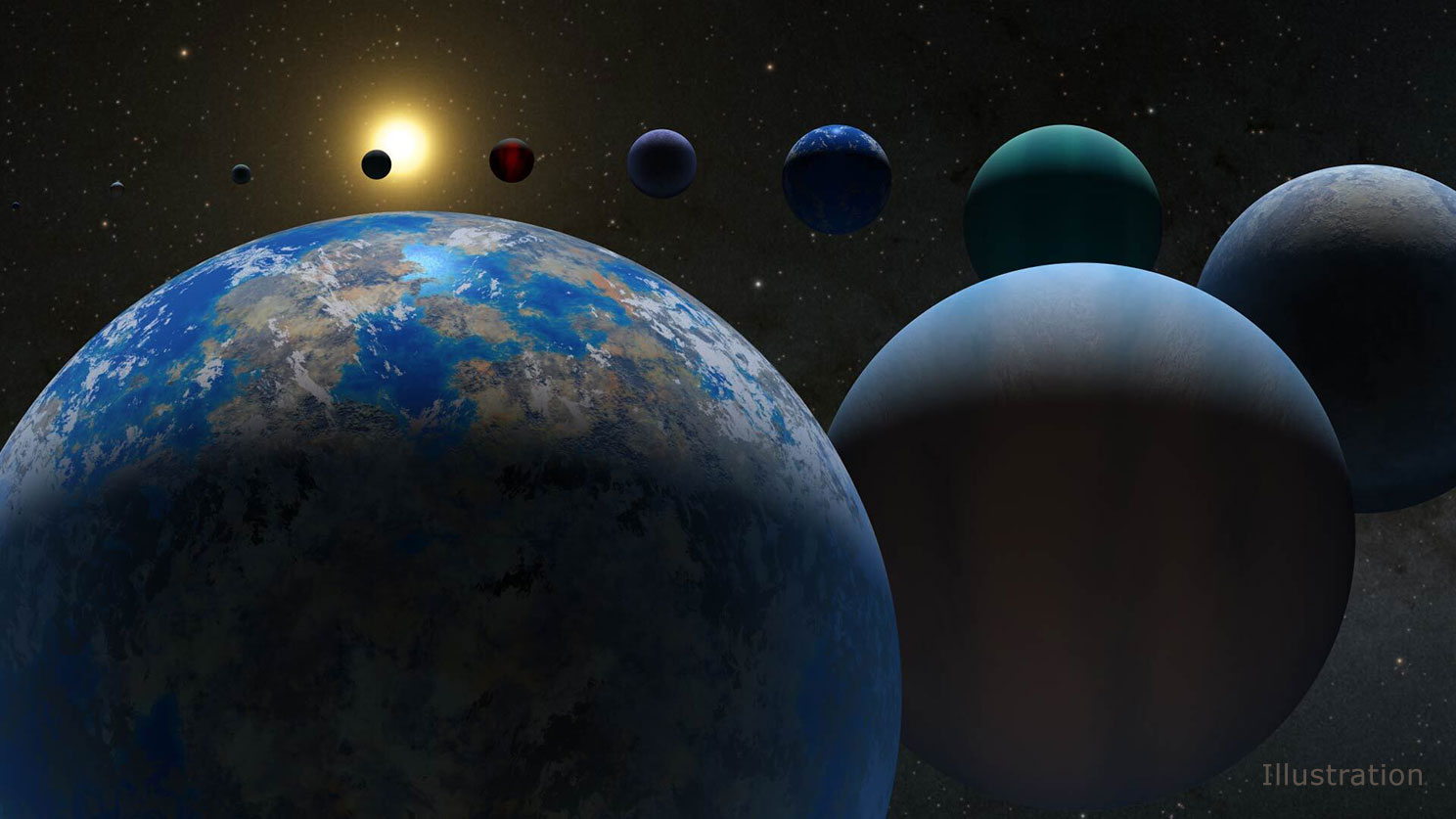
- Although it took us until the 1600s to piece it together, we eventually learned that our Solar System was heliocentric, with inner, rocky planets and outer, gas giant worlds.
- Although new discoveries ensued, including asteroids, additional planets, and a Kuiper belt, most held the expectation that planets around other stars — exoplanets — would follow similar configurations.
- It’s now 2024, and surprise after surprise has shown us that our own Solar System is anything but typical. It’s a stark reminder of how diverse the Universe truly can be.
When you look to the glittering points of light in the night sky — the stars visible to the naked eye — do you wonder, as so many have before you, about the planets that orbit around them, and what types of conditions they have on them? How many worlds do they have, and are they rocky, icy, or gas-dominated in nature? What types of elements and chemical compounds are abundant on them, and do they have atmospheres, precipitation, and liquids on or beneath their surfaces? And, like we see on Earth, is it possible that there are life forms on any of those worlds, and if so, how are they similar to or different from life on our own planet?
Back in the year 1600, Giordano Bruno was infamously burned at the stake for asking such questions and suggesting creative answers; just a few years later, Johannes Kepler and Galileo Galilei would overthrow the geocentric picture of our Solar System and demonstrate that the Earth was not stationary and unmoving, but rather revolved around the Sun like any of the other planets. As time progressed, additional discoveries, like the law of gravitation, new planets, asteroids and periodic comets, gave us expectations for what other stellar and planetary systems should look like. Yet, the overwhelming majority of them are nothing like our own. Here’s how coming to understand our own Solar System led planet-hunters astray.
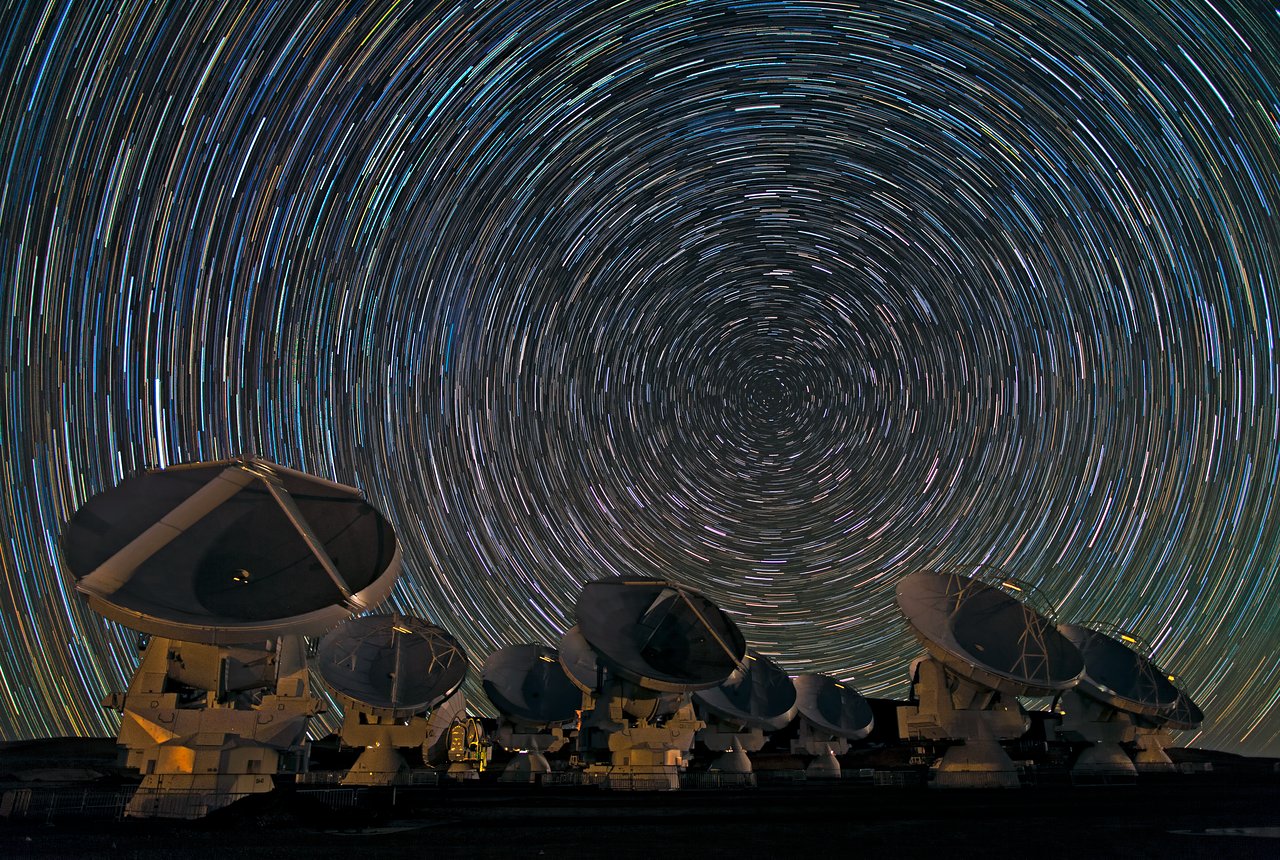
Contrary to what you might think, it wasn’t “obvious” that our Solar System was heliocentric and not geocentric. Consider that there were only three obvious pieces of evidence one could point to about the nature and shape of the Solar System:
- the daily (apparent) rotation of the entire sky, including all heavenly objects beyond Earth’s atmosphere,
- the annual cycling of the seasons, where the Sun appears to rise high (summer solstice) and then transition to low (winter solstice) and back again, with a period of one calendar year,
- and the anomalous motion of the planets, or wanderers of the night sky, with respect to the fixed stars.
The Moon very clearly revolved around the Earth once per month, and the other three points all had two explanations. The sky could rotate once every 24 hours because the Earth was rotating, or it could rotate because the heavens themselves (and everything in them) rotated once every 24 hours. The seasons could cycle because the Earth revolved around the Sun while tilted on its axis, or they could cycle because the Sun, orbiting the Earth daily, would actually modulate its motion in the up-and-down direction on an annual basis. And the planets could wander because they were on their own heliocentric orbits around the Sun, or their wandering could be explained by epicycles within a geocentric framework.
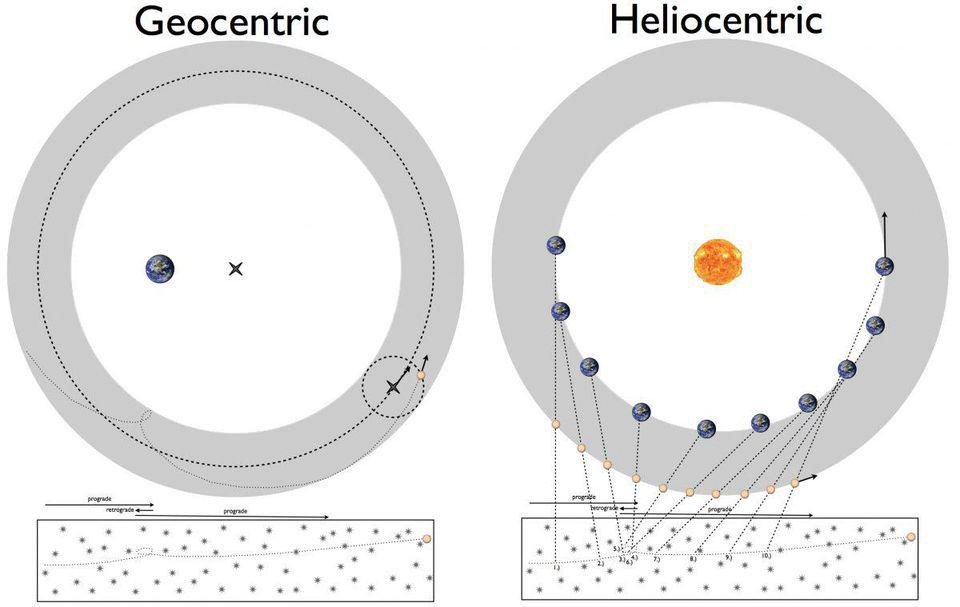
When you have multiple possible explanations for a set of observed phenomena, the key scientific test you need to perform is to identify predictions that differ between the two plausible explanations. Both Kepler and Galileo did this, but went about it in vastly different ways.
For Galileo, the key advance was the invention and use of the telescope for astronomical purposes. He looked at Jupiter, and noticed first three, then four little pinpricks of light near it, that could be seen moving around Jupiter over time. These four Galilean Moons — Io, Europa, Ganymede, and Callisto — were clearly orbiting an object other than the Earth, and this evidence was clear and overwhelming evidence against an “everything is geocentric” perspective.
But an even stronger piece of evidence came by observing Venus through a telescope. Unlike Jupiter and Saturn, which Galileo could also resolve, Venus didn’t always remain in a “full” or “nearly full” phase, but was exhibited to run through the full gamut of phases: from crescent to half-full to gibbous to full and then back again until a new (or nearly new) phase. Moreover, Venus clearly made close approaches to Earth during the crescent phases, and was distant during the full and nearly full phases. If Venus and the Sun both orbited the Earth, rather than Venus and Earth both orbiting the Sun, these observations would be a physical impossibility.
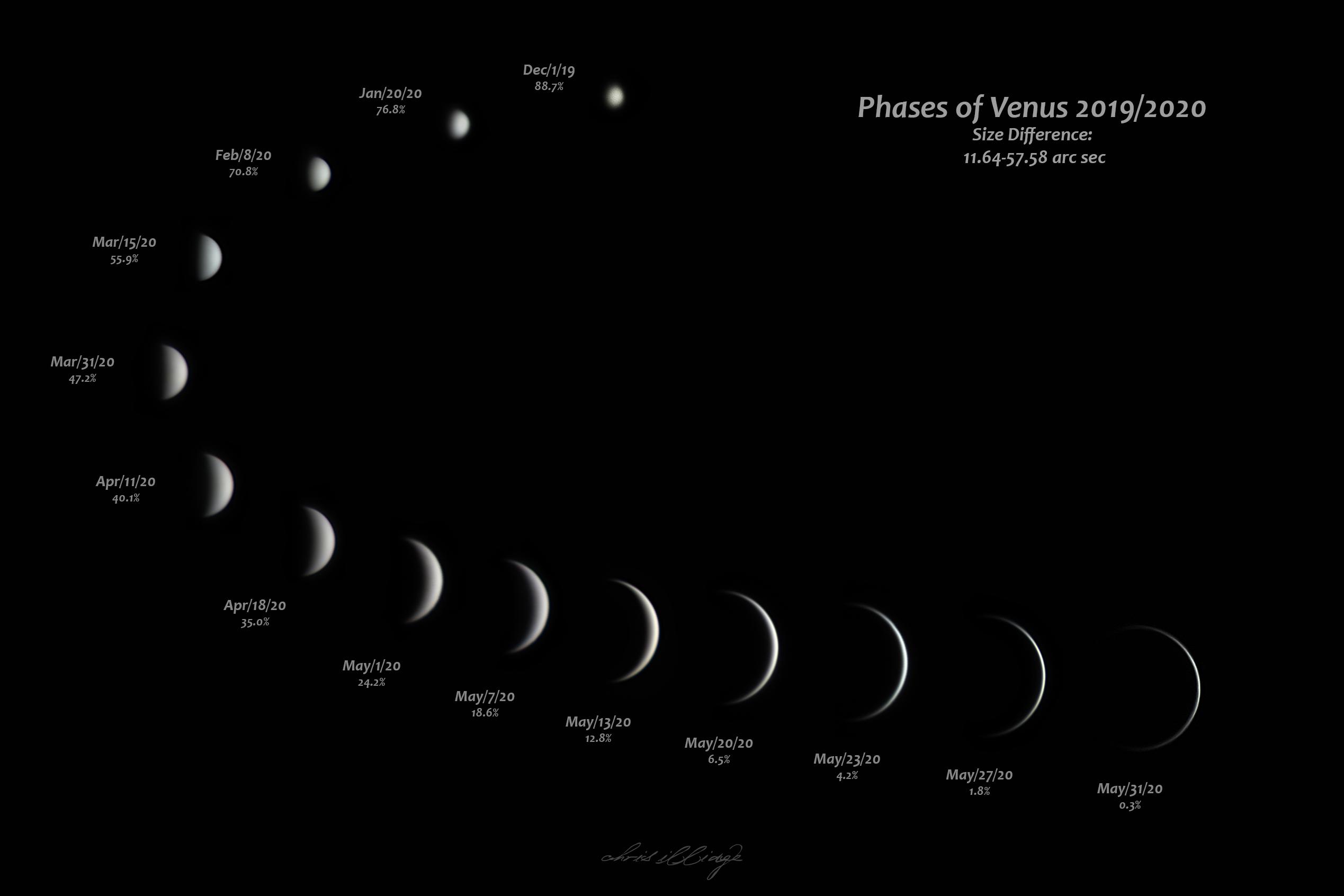
Kepler, on the other hand, primarily relied on Tycho Brahe’s precise observations of the planets, and in particular, of the positions of the planet Mars. Of all the planets, Mars’s orbit was the most puzzling, as both Ptolemy’s geocentric model and Copernicus’s original heliocentric model (with circular orbits and, to account for the observed mismatch, epicycles as well) failed to match the model’s predictions. Kepler himself had previously put forth his own heliocentric model with circular orbits (sans epicycles), but they all faced the same difficulties: when it came to the orbit of Mars, none of the models perfectly matched what was observed.
But Kepler, in a stroke of brilliance, did away with the notion of circular orbits and instead considered replacing them with elliptical ones: with the Sun located at one focus of the ellipse, rather than at the center of a circle. Immediately, the math of the predicted orbits matched up with the observations better than any model ever had before. With one fell swoop, Kepler could make better predictions about the past and future motions of the planets than ever before, and even devised three laws of planetary motion that quantitatively described the motion of the planets, their speed in orbit, and the relationship between their orbital periods and their distances from the Sun. After more than two millennia, the science of the Solar System was finally beginning to mature.

In subsequent generations, many more advances were made, improving our knowledge of our own cosmic backyard. Isaac Newton developed the inverse square law of universal gravitation, giving an underlying physical mechanism that applied equally well to objects on Earth as it did to explaining the motion of planetary bodies in our Solar System. In fact, all three of Kepler’s laws can be derived, in a relatively straightforward (although not necessarily an easy) fashion from Newton’s law of universal gravitation. Around the same time, Edmond Halley put forth the idea of periodic comets: another consequence completely in line with Newton’s laws and theories.
In the 1700s, William Herschel discovered Uranus: the Solar System’s seventh planet. Beginning in the early 1800s, numerous additional objects were discovered lying between the orbits of Mars and Jupiter: the first being Ceres, discovered by Giuseppe Piazzi. Today, thousands of bodies are known to exist in that region, defined as our Solar System’s asteroid belt. In 1846, tiny deviations in the orbit of Uranus (noted as conflicts with Kepler’s 2nd law) led to a prediction and then discovery of an eighth planet: Neptune. And beyond Neptune, first Pluto, then Pluto’s moon Charon, and finally several other Kuiper belt objects were discovered in the 20th century. It led to an impressive picture of our Solar System, at last.
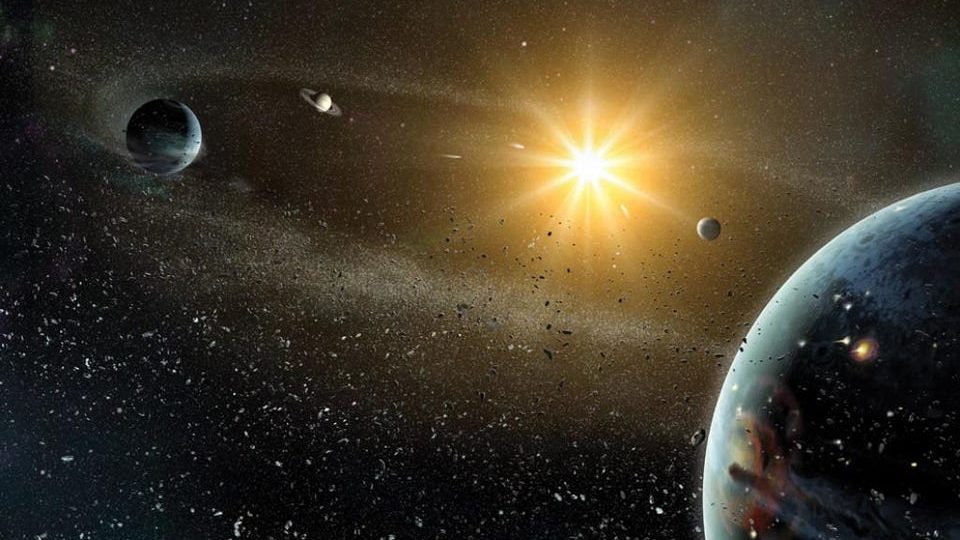
One would think, then, that if we were going to look for planets around other stars — what we know today as exoplanetary systems — that the smart move would be to use our own Solar System’s structure as a template for finding planets around other stars. To recap, starting from the center and moving outward, our Solar System’s structure is:
- a single, massive star that’s much more massive than any of the planets,
- followed by small, low-mass, rocky worlds in the interior,
- with an asteroid belt that lies beyond the last one,
- followed by a series of massive, distant, slow-orbiting gas giant planets,
- which then gives way to an outer belt of icy objects,
- and with potentially even more low-mass, ice-rich objects beyond that in a spheroidal cloud-like configuration.
How would we go about finding, detecting, and characterizing these worlds? Although there are many methods, three of them were promising, simple, and relatively straightforward.
The first successful exoplanet detection method was the stellar wobble method, where a star would be closely monitored to look for periodic small motions, as Jupiter, the most massive planet in the Solar System, causes the Sun to not remain still, but rather to orbit the Solar System’s barycenter (or center of mass). While the side-to-side motions are not detectable, the back-and-forth motions along any observer’s line-of-sight could, if our instruments were sensitive enough, reveal the presence and orbital period of any sufficiently massive (relative to the mass of its parent star) planetary companion. (Note: the “stellar wobble method” was the original name for this method, but “radial velocity method” has become more common in recent years.)
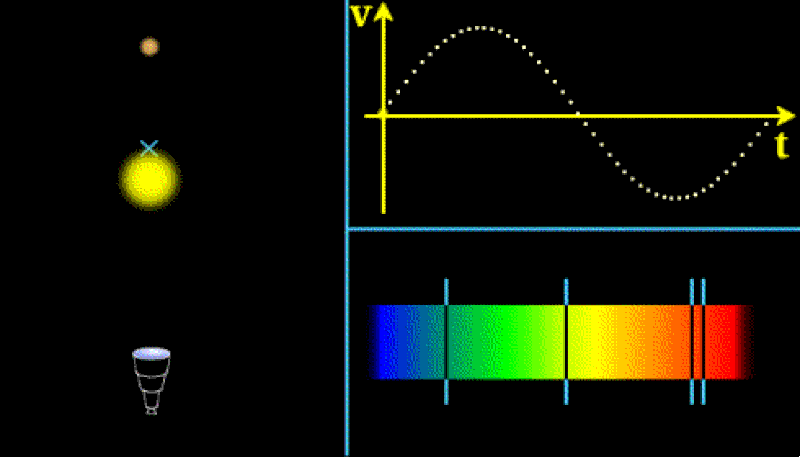
But when the planets first detected with this method came back, they sent shockwaves through the astronomy and planetary science communities. They weren’t like the planets in our Solar System at all, but rather were large, massive, and extremely close to their parent stars: a class of exoplanets known as hot Jupiters. Unlike our own Solar System, it seems like giant planets actually can exist in tight, short-period orbits around their parent stars. Not every stellar system was like our own.
Next, the transit method for exoplanet detection would pay dividends. Again, simply by monitoring the parent star, you could use properties of the starlight detected over time to determine the presence of at least a subset of the planets that orbited it. In this case, you’d look at:
- one or more stars,
- over long periods of time,
- and look for periodic dimming,
- that would be consistent with a spherical body, like a planet, passing in front of the star,
- and blocking a fraction of its light during these “transit” events.
Planet-finding missions, like NASA’s Kepler and TESS, have found more exoplanets than any other method leveraging this technique. To date, of the more than 5000 exoplanets that are known, well over half of them were identified with the transit method.
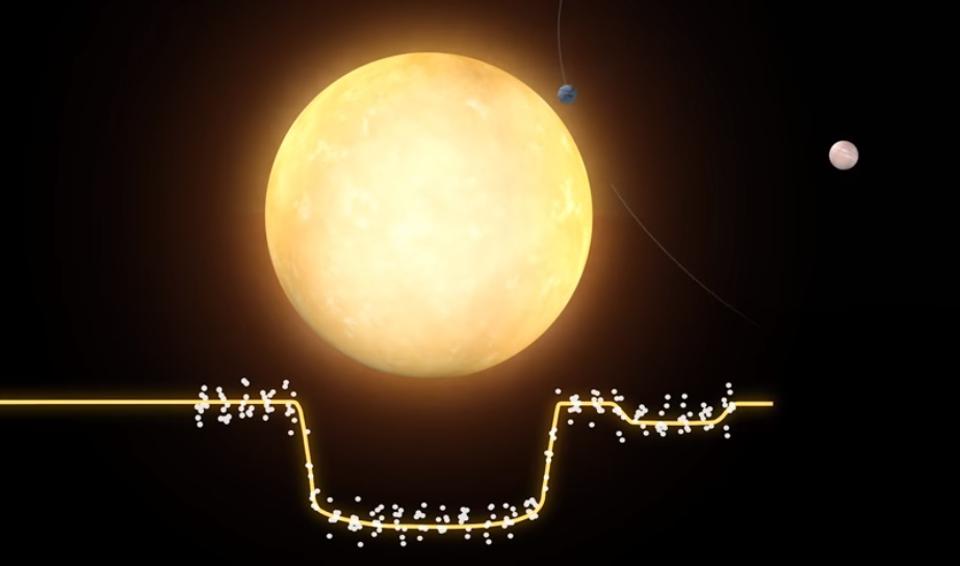
But again, there were tremendous surprises. Of the exoplanets that were discovered:
- most of them were short-period planets, indicating their relative closeness to their parent stars,
- they came in all varieties of sizes, but the most common size isn’t represented at all in our Solar System: mini-Neptune worlds, between about two and ten Earth masses,
- and of the systems with multiple known exoplanets, very few of them had both small, low-mass, rocky worlds and gas giant worlds as well.
Whereas, in ~1990 or before (prior to the discovery of the first exoplanets around normal, Sun-like stars), we once thought that our Solar System’s configuration would be typical, we’ve now learned that there’s an enormous variety of planetary systems out there, and our configuration is just one of many.
However, if we’re looking for very distant giant planets, there’s a third method that’s more successful than either the stellar wobble or the transit method: direct imaging. Advances in coronagraphy — where the bright light from the parent star is obscured, enabling the possibility of detecting either reflected starlight or (infrared) internally generated heat from a giant planet — have shown us that at much greater distances than any of the planets are located at in our own Solar System, there are abundant populations of giant, Jupiter-like worlds located at extreme distances from their parent stars, well beyond the edge of our known Kuiper belt.

And yet, one can ask the following question: if we were to place a “twin” of our Solar System a relatively short distance away, like ~100 light-years or so, what would these various methods, using the pinnacle of modern technology, have revealed to us?
The answer, shockingly, is no planets at all.
Compared to the Sun, the Earth is tiny, and capable of blocking under ~0.01% of the Sun’s light: an insufficient amount to detect it with the transit method. The gas giant worlds are too far away and take too long to complete a single orbit for the stellar wobble method to reveal their presence. (We would need to monitor the Sun for 12 years to detect even one complete Jupiter orbit.) And even the most distant large planet, Neptune, is only ~30 A.U. (thirty times the Earth-Sun distance) away from the Sun, meaning that it’s too small and too faint, at that distance, to be directly imaged at all.
Instead, the exoplanets that we’ve found have showcased a combination of two things:
- what is actually out there,
- and what types of planets our current detection technology is able to reveal.
It’s a sobering message from the Universe, to realize that even with all we’ve learned about the thousands of stars and star systems now known to possess planets, that absolutely zero of them even had a chance of being Solar System-like.
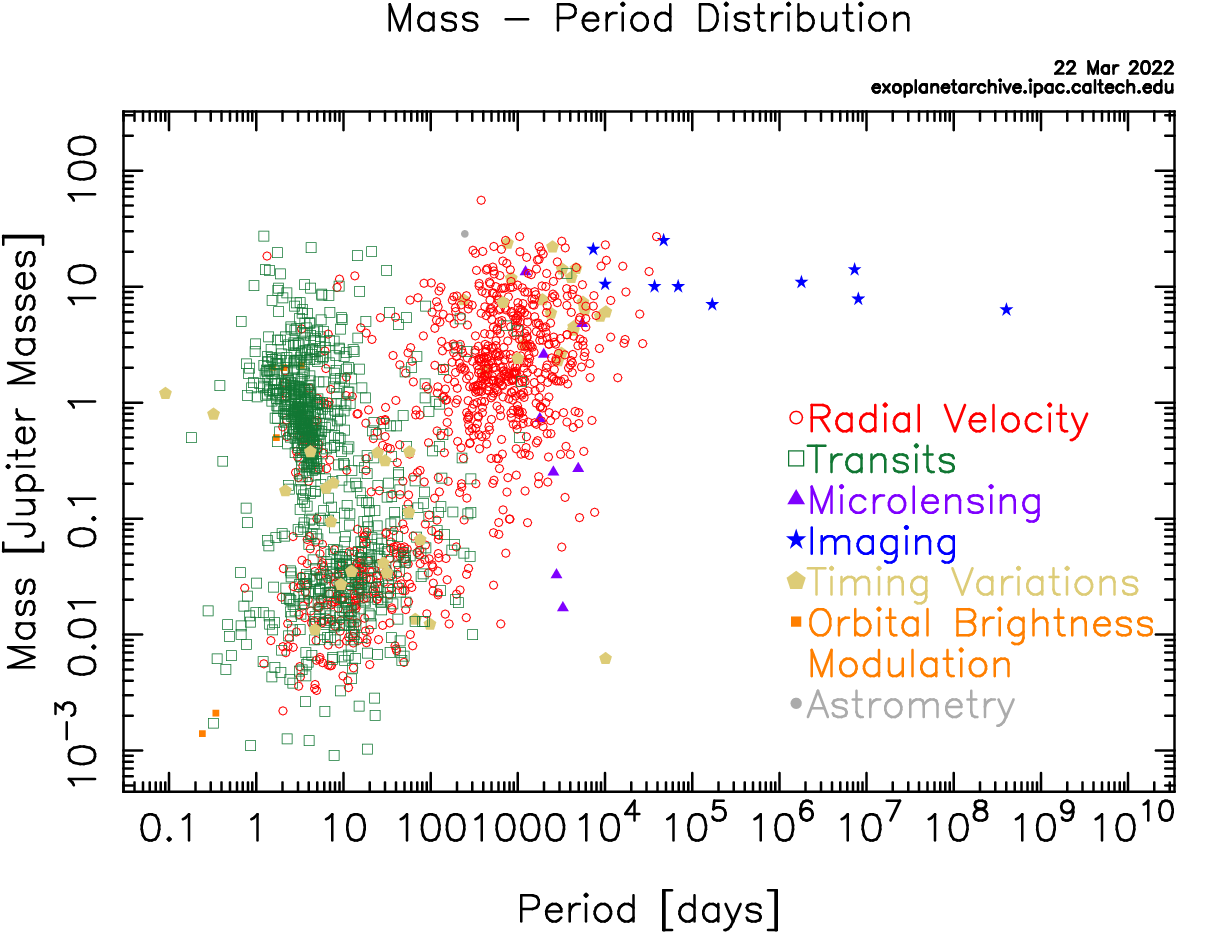
Putting all of the pieces together, we’ve now unveiled three main classes of exoplanets:
- massive, Jupiter-like worlds located at Earth-like or greater distances from their stars,
- massive, Jupiter, Saturn, Neptune, and mini-Neptune-like worlds that closely orbit their parent stars,
- and small, terrestrial, rocky worlds that closely orbit their low-mass, small, faint (predominantly red dwarf) parent stars.
Of course, none of these apply to any of the planets in our Solar System, and that’s a good thing: if every stellar system were like our own Solar System, we wouldn’t have successfully detected all of the exoplanets we now know about!
However, with upcoming observatories like NASA’s Habitable Worlds Observatory and ground-based 30-meter class telescopes like the GMT and the ELT, it’s finally going to arrive: the era where we will be sensitive to Earth-sized planets at Earth-like distances around Sun-like stars. We have every reason to expect that there will be examples that are very much like us out there, but no right to expect that planetary systems like our own are the majority, the plurality, or even common. There’s an enormous diversity of planets and planetary systems out there in the Universe, and our Solar System is only one isolated example. It just happens to be our own.